Quantum Locking
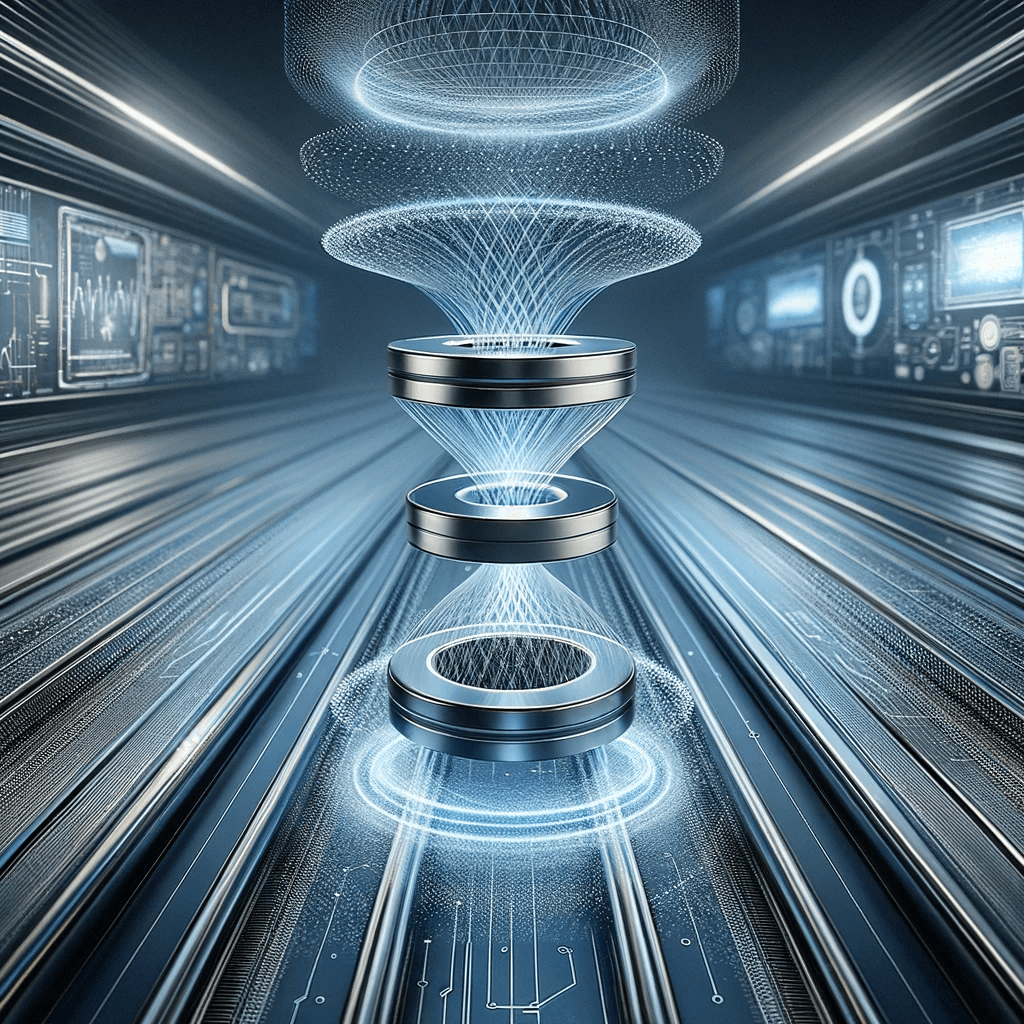
Quantum locking, a phenomenon encapsulating the most profound elements of quantum mechanics and superconductivity, has been subject to much intrigue and scientific exploration since its discovery. This breakthrough innovation, tied intrinsically to the unique properties of superconductors, offers the world glimpses into a future with possibilities currently beyond our conventional scientific understandings.
A superconductor, when cooled below a critical temperature, exhibits a fascinating characteristic of carrying electric current without any electrical resistance. Moreover, it showcases the Meissner effect, which means it can expel magnetic fields from within itself. However, this is not an absolute property. In type-II superconductors, when exposed to a magnetic field stronger than a certain threshold, they permit some magnetic field lines to infiltrate their interior, thereby creating what is referred to as quantized vortices.
In this setup, when one attempts to move the superconductor or alter the magnetic field, the positions of the quantized vortices also change. However, this change demands energy due to the interaction between the vortices and the superconductor’s crystal lattice, a phenomenon recognized as pinning. The consequence of this is that the superconductor gets “locked” in place relative to the magnetic field, an occurrence termed as quantum locking or flux pinning.
- The phenomenon of quantum locking was brought to mainstream attention by a 2011 video from the Association of Science-Technology Centers (ASTC) annual conference. In the video, a superconductor locked in a magnetic field demonstrates levitation and retains its position even when the magnetic field is manipulated (Source: Association of Science-Technology Centers (ASTC)).
- Quantum locking may have significant implications for the design of future transportation systems. Magnetic levitation (maglev) trains could be enhanced by this technology, creating transportation that is fast and almost entirely frictionless. However, these potential applications are still in their nascent stages (Source: Journal of Applied Physics).
- Quantum locking could offer breakthroughs in the field of energy storage. Superconductors can carry an electric current indefinitely when cooled to their critical temperature, which can be harnessed for zero-loss energy storage. This property, combined with quantum locking, could lead to innovations in grid-scale energy storage (Source: Nature Energy).
Physicist Dr. Boaz Almog from Tel Aviv University, have demonstrated quantum locking and suggested its potential applications. Almog’s work has brought to light the ways this phenomenon could revolutionize numerous sectors, including transportation and energy storage.
“Introduction to Solid State Physics” by Charles Kittel, a highly regarded textbook, offers a comprehensive discussion on the subject of superconductors and by extension, discusses quantum locking. The book underscores the significance of understanding this phenomenon and its implications on the future of physics and technology.
One of the chief challenges faced in making quantum locking a universally applicable phenomenon is maintaining superconductors at extremely low temperatures, required for them to maintain their superconducting state. The future of quantum locking hinges on advancements in the field of materials science that could potentially result in superconductors that work at room temperatures.
Quantum locking indeed represents a paradigm shift in our understanding of material science and quantum physics. It exemplifies how sophisticated our technological and scientific advancements have become. Still, there is a significant amount of research and development required before this phenomenon can be implemented in daily applications.
One of the main challenges is that quantum locking requires superconductors, materials that exhibit zero electrical resistance and expel magnetic fields when cooled below a certain critical temperature. Most known superconductors require extremely low temperatures, often near absolute zero, to maintain their superconducting state. Maintaining these temperatures is energy-intensive and currently impractical for widespread use.
Superconductivity arises when electrons in a material form pairs, called Cooper pairs, and these pairs move through the lattice structure of the material without scattering off impurities or lattice vibrations (phonons). This lack of scattering leads to zero electrical resistance.
This pairing is a quantum mechanical effect, and it’s a delicate balance. It requires a certain energy gap between the paired state and the normal, unpaired state. In most materials, thermal energy from heat is more than sufficient to break these pairs apart. As temperature increases, the thermal energy of the electrons also increases, causing the Cooper pairs to break apart and thus destroying the superconducting state.
Therefore, for a material to exhibit superconductivity, it usually needs to be cooled to a temperature where thermal vibrations are minimal, so the Cooper pairs can form and persist. For most known superconductors, this critical temperature is extremely low, often close to absolute zero.
The main categories of superconducting materials include:
- Elemental Superconductors: These are simple elements from the periodic table that become superconducting at very low temperatures. Examples include mercury (the first element discovered to be superconducting), lead, and niobium.
- Alloys and Metallic Compounds: Some combinations of metals can become superconducting. For example, niobium-tin (Nb3Sn) and niobium-titanium (NbTi) are widely used in superconducting magnets due to their relatively high critical temperature and high critical magnetic field.
- Ceramic or “High-Temperature” Superconductors: These materials, often based on a planar structure of copper-oxide layers, can become superconducting at higher temperatures than elemental superconductors or metallic superconductors (though still very cold by everyday standards). The most well-known high-temperature superconductor is probably YBa2Cu3O7 (Yttrium-Barium-Copper-Oxide, or YBCO), which becomes superconducting below about -181 degrees Celsius (-294 degrees Fahrenheit).
- Iron-Based Superconductors: Discovered in 2006, these are a newer class of high-temperature superconductors. They often have a layered structure similar to the ceramic superconductors, but with iron atoms playing a crucial role.
Each of these materials has its own challenges and advantages. For example, while ceramic superconductors can operate at higher temperatures, they are generally brittle and difficult to shape into wires or other useful forms. NbTi and Nb3Sn, on the other hand, are ductile and can be made into wires, but they require very low temperatures.
The study of quantum locking is not just about developing cool gadgets or transforming transportation. It’s about expanding our understanding of the universe and the fundamental laws of physics. It provides insights into the quantum world, an area still fraught with mysteries and unanswered questions.